Cellular Respiration
Discover the intricacies of cellular respiration, a critical process for energy production in cells, encompassing aerobic and anaerobic pathways and their molecular mechanisms
Key Takeaways
- Cellular respiration is a vital biological process for energy production in cells.
- It involves converting nutrients into ATP, the primary energy currency.
- Two types: Aerobic (uses oxygen) and Anaerobic (without oxygen).
- Key components: Mitochondria in eukaryotes, cytoplasm in prokaryotes.
- Stages of Aerobic Respiration: Glycolysis, Citric Acid Cycle, Oxidative Phosphorylation.
Welcome to our in-depth exploration of cellular respiration—an essential process responsible for energy production in living organisms. In this blog, we'll uncover the ins and outs of both aerobic and anaerobic respiration, explaining their stages and how they work on a molecular level. Along this scientific journey, we'll highlight the importance of aerobic respiration, which relies on oxygen to get the most energy from nutrients, and explore the interesting world of anaerobic respiration, which comes into play when there's limited oxygen but the need for energy remains. Additionally, we'll delve into the Warburg effect, a fascinating concept in cancer research that reveals changes in cellular metabolism. Whether you're a biology enthusiast, a curious student, or a researcher in the field of cellular energy, join us as we unravel the mysteries of cellular respiration, grasp its crucial role in sustaining life, and understand its relevance in cancer biology.
Table of Contents
Jump to a section:
What is Cellular Respiration?
Cellular respiration is a fundamental biological process through which cells generate energy by converting nutrient molecules into adenosine triphosphate (ATP), the primary energy currency of cells. It is a highly efficient and intricate metabolic pathway that occurs in the mitochondria of eukaryotic cells, as well as in the cytoplasm of prokaryotic cells. The process involves the breakdown of glucose and other organic molecules in the presence of oxygen (aerobic respiration) or, in the absence of oxygen, the breakdown of glucose via other pathways like fermentation (anaerobic respiration). Through cellular respiration, cells release stored energy, which fuels essential cellular activities such as muscle contractions, active transport of molecules, synthesis of macromolecules, and the maintenance of cellular processes.
Overview of Cellular Respiration in Eukaryotes
Key players of Cellular Respiration - Mitochondria and ATP
Mitochondria and adenosine triphosphate (ATP) are central to the process of cellular respiration, orchestrating the efficient production and utilization of energy within cells. Mitochondria, often referred to as the "powerhouses of the cell," are double-membrane-bound organelles found in eukaryotic cells. These tiny structures play a crucial role in aerobic respiration, housing many of the enzymes and transport proteins involved in the process. They facilitate the breakdown of glucose and other organic molecules, enabling the extraction of high-energy electrons and protons, which are then used to generate ATP.
ATP, on the other hand, is the energy carrier molecule that fuels cellular processes. It stores and transfers energy in the form of high-energy phosphate bonds. During cellular respiration, ATP is produced through oxidative phosphorylation, a process that takes place in the inner mitochondrial membrane in eukaryotic cells. Here, the electrons and protons derived from the breakdown of glucose are shuttled through the electron transport chain (ETC), which creates an electrochemical gradient across the inner membrane. This gradient drives ATP synthase to produce ATP by adding a phosphate group to adenosine diphosphate (ADP).
Cellular Respiration in Prokaryotes
Interestingly, in prokaryotic cells, which lack mitochondria, cellular respiration takes place in the cell's cytoplasm. The electron transport chain is located in the cell membrane, and the protons are pumped across this membrane to create the electrochemical gradient necessary for ATP synthesis. Prokaryotes have evolved diverse strategies to carry out cellular respiration effectively, allowing them to thrive in various environments. Despite the differences in cellular organization, the reliance on ATP as the universal energy carrier remains a common feature across all living organisms, underscoring the essential role of both mitochondria and ATP in the intricate dance of cellular respiration.
Aerobic Respiration
Aerobic respiration is a sophisticated and highly efficient metabolic process that fuels the majority of cellular energy production in the presence of oxygen. Taking place within the mitochondria of eukaryotic cells, aerobic respiration serves as the cornerstone of energy extraction, allowing cells to harness the full potential of nutrient molecules, particularly glucose.
Stages of Aerobic Respiration
Aerobic respiration is a complex process involving three main stages: glycolysis, the citric acid cycle (also known as the Krebs cycle or TCA cycle), and oxidative phosphorylation (including the electron transport chain). Let's explore each stage in detail
Glycolysis
Glycolysis is the initial stage of aerobic respiration and occurs in the cytoplasm of the cell. It is the common pathway for both aerobic and anaerobic respiration, meaning it can occur with or without the presence of oxygen. In glycolysis, one molecule of glucose (a 6-carbon sugar) is broken down into two molecules of pyruvate (a 3-carbon compound).
Once Glucose from the bloodstream enters the cell through different glucose transporters, it is activated by hexokinase, consuming one ATP molecule and converting it into glucose-6-phosphate. An isomerase enzyme, phosphoglucose isomerase, then converts glucose-6-phosphate into fructose-6-phosphate. Following this, another ATP molecule is used to phosphorylate fructose-6-phosphate, forming fructose-1,6-bisphosphate, with the enzyme phosphofructokinase-1 (PFK-1) catalyzing the reaction. Fructose-1,6-bisphosphate is then cleaved into two 3-carbon compounds: dihydroxyacetone phosphate (DHAP) and glyceraldehyde-3-phosphate (G3P) by aldolase. Triose phosphate isomerase then converts one molecule of DHAP into another molecule of G3P. Each G3P molecule is then oxidized by glyceraldehyde-3-phosphate dehydrogenase (GAPDH), and NAD+ gains two electrons and a hydrogen ion to form NADH, while simultaneously, one phosphate group is transferred to ADP, generating ATP and resulting in the production of two molecules of 1,3-bisphosphoglycerate. In the next step, each 1,3-bisphosphoglycerate donates its phosphate group to ADP, forming ATP and converting into 3-phosphoglycerate with the enzyme phosphoglycerate kinase (PGK) catalyzing the reaction. Subsequently, the enzyme phosphoglycerate mutase converts 3-phosphoglycerate into 2-phosphoglycerate. 2-phosphoglycerate undergoes dehydration with enolase, leading to the formation of phosphoenolpyruvate (PEP). PEP then donates its phosphate group to ADP, forming ATP, and is transformed into pyruvate with the enzyme pyruvate kinase (PK) catalyzing the reaction. This completes the glycolysis pathway, producing two molecules of pyruvate, two molecules of NADH, and a net gain of two ATP molecules per glucose molecule.
Pyruvate Decarboxylation
Pyruvate decarboxylation is a critical step in cellular respiration that occurs after glycolysis and before the citric acid cycle. During this process, each molecule of pyruvate (a 3-carbon compound) generated from glycolysis undergoes enzymatic decarboxylation, resulting in the removal of a carbon dioxide (CO2) molecule. The purpose of pyruvate decarboxylation is to prepare the pyruvate for entry into the citric acid cycle (also known as the Krebs cycle) and to yield acetyl-CoA, a two-carbon compound that carries high-energy electrons.
The decarboxylation of pyruvate involves a multienzyme complex called the pyruvate dehydrogenase complex (PDC), which is located in the mitochondria of eukaryotic cells. The PDC consists of three main enzymes: pyruvate dehydrogenase (E1), dihydrolipoamide transacetylase (E2), and dihydrolipoamide dehydrogenase (E3).
The overall result of pyruvate decarboxylation is the conversion of one molecule of pyruvate into one molecule of acetyl-CoA, as well as the release of one carbon dioxide molecule.
The Citric Acid Cycle (Krebs Cycle)
The pyruvate generated from glycolysis enters the mitochondria, where it undergoes further breakdown in the citric acid cycle. This cycle occurs in the mitochondrial matrix and completes the oxidation of glucose. For each glucose molecule, the citric acid cycle occurs twice (one cycle for each pyruvate). The steps of the citric acid cycle are as follows:
After pyruvate decarboxylation, acetyl-CoA combines with oxaloacetate to form citrate, a 6-carbon compound, catalyzed by citrate synthase. Citrate then undergoes isomerization by aconitase to form isocitrate. In the first oxidative decarboxylation, isocitrate is oxidized by isocitrate dehydrogenase, reducing NAD+ to NADH and producing α-ketoglutarate, while releasing a carbon dioxide molecule. α-ketoglutarate undergoes a second oxidative decarboxylation, catalyzed by α-ketoglutarate dehydrogenase complex, reducing NAD+ to NADH and releasing another carbon dioxide molecule, resulting in the formation of succinyl-CoA. Substrate-level phosphorylation occurs when succinyl-CoA donates its phosphate group to ADP, catalyzed by succinyl-CoA synthetase, producing one ATP molecule and converting into succinate. Succinate is oxidized by succinate dehydrogenase, reducing FAD to FADH2, forming fumarate. Fumarate undergoes hydration by fumarase to become malate, which is then oxidized by malate dehydrogenase, converting NAD+ to NADH and generating oxaloacetate, the starting compound for the next round of the citric acid cycle. The citric acid cycle, also known as the Krebs cycle, serves as a critical pathway for extracting high-energy electrons from acetyl-CoA, further fueling cellular energy production through the electron transport chain and oxidative phosphorylation.
Overview of Glycolysis and Krebs Cycle in an eukaryotic cell
Oxidative Phosphorylation
The final stage of aerobic respiration is oxidative phosphorylation, which occurs in the inner mitochondrial membrane. It involves two key components: the electron transport chain (ETC) and chemiosmosis:
1. Electron Transport Chain (ETC): The NADH and FADH2 generated in glycolysis and the citric acid cycle donate their high-energy electrons to the ETC. These electrons pass through a series of protein complexes, known as Complex I, II, III, and IV, embedded in the inner mitochondrial membrane. The electrons transfer energy to pump protons (H+) from the mitochondrial matrix to the intermembrane space, establishing an electrochemical gradient.
2. Chemiosmosis: The electrochemical gradient created by the ETC is harnessed by ATP synthase, an enzyme complex embedded in the inner mitochondrial membrane. As protons move back into the mitochondrial matrix through ATP synthase, ADP and inorganic phosphate combine to form ATP. This process is referred to as chemiosmosis and accounts for the majority of ATP generated during aerobic respiration.
In the final step of oxidative phosphorylation, the electrons, after passing through the ETC, combine with oxygen and protons to form water, which is the final electron acceptor in aerobic respiration.
Electron Transport Chain Diagram
Explore our Metabolism Assays
ELISA Kits related to Respiration
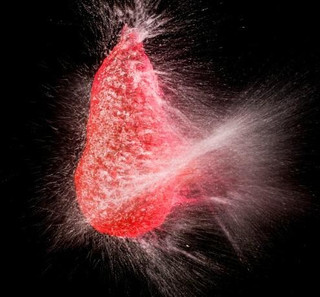
Adenosine triphosphate (ATP) ELISA Kit | |
---|---|
ELISA Type: | Competitive |
Range: | 1.56-100 ng/ml |
Reactivity: | General |
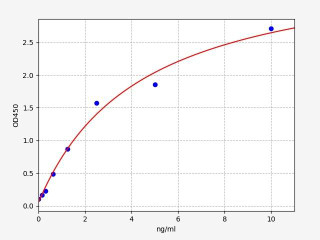
Human Pyruvate Dehydrogenase Alpha / PDHA ELISA Kit | |
---|---|
ELISA Type: | Sandwich ELISA, Double Antibody |
Sensitivity: | 0.094ng/ml |
Range: | 0.156-10ng/ml |
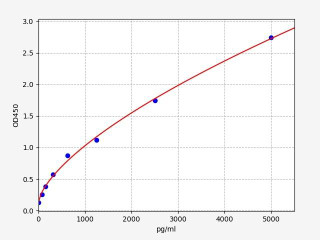
Human HK1 (Hexokinase-1) ELISA Kit | |
---|---|
ELISA Type: | Sandwich ELISA, Double Antibody |
Sensitivity: | 46.875pg/ml |
Range: | 78.125-5000pg/ml |
Anaerobic Respiration
Anaerobic respiration is a type of cellular respiration that occurs in the absence of oxygen or when oxygen levels are limited. It is an alternative metabolic pathway to aerobic respiration, enabling cells to produce energy in environments where oxygen is scarce. Despite its lower energy yield compared to aerobic respiration, anaerobic respiration allows certain organisms, including some bacteria and yeast, to survive and carry out essential cellular functions in oxygen-deprived conditions. In anaerobic respiration, alternative electron acceptors, such as nitrate or sulfate, are used in place of oxygen, leading to the partial breakdown of glucose or other organic molecules. This process is particularly relevant in oxygen-limited environments such as the deep layers of soil, aquatic sediments, and the guts of certain animals.
Lactate Fermentation
One of the most well-known forms of anaerobic respiration is lactate fermentation. In this process, which occurs in some microorganisms and animal cells, pyruvate, the end product of glycolysis, undergoes a reduction reaction in the absence of oxygen. Pyruvate is converted into lactate, a three-carbon compound, by the enzyme lactate dehydrogenase. This reaction serves to regenerate NAD+ from the NADH generated during glycolysis. By replenishing NAD+, glycolysis can continue to produce ATP, albeit at a lower rate than aerobic respiration. Lactate fermentation is associated with temporary muscle fatigue in humans during intense physical activities, where oxygen demand surpasses supply, leading to a build-up of lactate in muscles. Over time, when sufficient oxygen becomes available, lactate can be converted back into pyruvate and metabolized through aerobic respiration.
Anaerobic Respiration - Lactic Acid Fermentation
Anaerobic Fermentation in Extremophiles
Certain bacteria are extremophiles, thriving in harsh environments with extreme temperatures, acidity, salinity, or lack of oxygen. For instance, some thermophilic bacteria inhabit high-temperature geothermal environments, while halophilic bacteria flourish in extremely saline habitats. These bacteria have evolved unique anaerobic respiration pathways to adapt to their extreme living conditions. For example, some anaerobic bacteria utilize sulfur compounds, like sulfate or sulfur, as electron acceptors instead of oxygen. This process produces hydrogen sulfide (H2S) or other sulfur compounds as byproducts. In other cases, nitrate-reducing bacteria can use nitrate (NO3-) as an alternative electron acceptor, generating nitrogen gas (N2) or nitrous oxide (N2O) as end products. The ability of these bacteria to perform anaerobic respiration allows them to thrive in environments unsuitable for many other life forms, shedding light on the extraordinary adaptability and diversity of microbial life on Earth.
How is Cellular Respiration Regulated?
Cellular respiration is precisely regulated to ensure efficient energy production and adaptability to the cell's dynamic needs. Regulation occurs at multiple levels, including substrate availability, oxygen availability, enzyme regulation through feedback inhibition and allosteric modulation, hormonal influence, redox potential, temperature, and pH. Substrate and oxygen availability directly influence the rate of cellular respiration, with enzymes playing a vital role in pathway control through feedback mechanisms. Hormones like insulin and glucagon modulate respiration by affecting nutrient availability, while the redox state and environmental factors such as temperature and pH also impact respiration rates. Additionally, cells monitor their ATP levels, adjusting respiration accordingly to meet energy demands. At the genetic level, transcriptional control influences the expression of enzymes, allowing the cell to adapt its metabolic capacity based on energy requirements and the cellular environment. This complex regulation ensures that cellular respiration is finely tuned for optimal ATP production and sustains cellular functions in diverse conditions.
Cellular Respiration and Photosynthesis
Cellular respiration and photosynthesis are interconnected through the cycling of energy and matter in living organisms. They form a vital biological relationship known as the "carbon cycle" and play complementary roles in maintaining the balance of energy and nutrients in ecosystems. Here's how cellular respiration is linked to photosynthesis:
1. Exchange of Gases: One of the most apparent links between cellular respiration and photosynthesis is the exchange of gases. During photosynthesis, plants and photosynthetic organisms take in carbon dioxide (CO2) from the atmosphere and release oxygen (O2) as a byproduct. This oxygen is essential for the process of cellular respiration in plants, animals, and other aerobic organisms, where oxygen serves as the final electron acceptor in the electron transport chain, leading to the synthesis of ATP.
2. Energy Flow: Photosynthesis harnesses solar energy to convert carbon dioxide and water into glucose and oxygen, storing the energy in the chemical bonds of glucose. This glucose then serves as a primary source of energy for living organisms, including plants themselves. During cellular respiration, glucose is broken down to release energy in the form of ATP, which fuels various cellular processes. The ATP generated in cellular respiration is used to drive essential functions like growth, movement, and reproduction in plants and other organisms.
3. Carbon Cycling: The carbon cycle is a key aspect of the link between photosynthesis and cellular respiration. During photosynthesis, carbon dioxide is incorporated into glucose and other organic molecules, storing carbon in plant tissues. When these plants undergo cellular respiration, the stored glucose is broken down, releasing carbon dioxide back into the atmosphere. The carbon dioxide released during cellular respiration is then available for uptake by plants during photosynthesis, closing the carbon cycle loop.
4. Energy Balance: Photosynthesis provides an influx of energy to ecosystems in the form of glucose and other organic molecules, while cellular respiration releases that energy through the breakdown of these molecules. This balance of energy flow is essential for sustaining life in ecosystems, where energy is continuously being converted and utilized by organisms.
5. Symbiotic Relationships: In some ecosystems, photosynthetic organisms and organisms carrying out cellular respiration may form symbiotic relationships. For instance, photosynthetic algae living within coral reefs provide oxygen and organic matter to support the respiration and energy needs of the coral polyps. This mutualistic interaction illustrates the close link between photosynthesis and cellular respiration in supporting the biodiversity and functioning of ecosystems.
Mitochondrial Dysfunction and Related Disorders
Mitochondrial dysfunction refers to abnormalities or impairments in the structure or function of mitochondria, the specialized organelles responsible for energy production within cells. Mitochondrial dysfunction can arise from various factors, including genetic mutations in mitochondrial or nuclear DNA, environmental influences, age-related changes, and exposure to toxins or certain medications. Since mitochondria are unique in having their own DNA, mutations in mitochondrial DNA can be particularly impactful in disrupting mitochondrial function. Dysfunctional mitochondria can have profound effects on cellular energy metabolism and overall cellular function, leading to various mitochondrial disorders and associated health conditions.
Mitochondrial disorders encompass a broad spectrum of conditions, which can be classified into different subtypes based on affected tissues, organs, or metabolic pathways. Some well-known mitochondrial disorders include Leigh syndrome, MELAS (mitochondrial encephalomyopathy, lactic acidosis, and stroke-like episodes), MERRF (myoclonic epilepsy with ragged red fibers), and Kearns-Sayre syndrome.
Mitochondrial dysfunction has also been implicated in the aging process and the pathogenesis of certain non-mitochondrial diseases, such as neurodegenerative disorders (e.g., Alzheimer's and Parkinson's diseases), cardiovascular diseases, diabetes, and some forms of cancer. The accumulation of mitochondrial DNA damage and the decline in mitochondrial function with age contribute to the aging phenotype and age-related diseases.
Warburg Effect
The Warburg effect was first described by Otto Warburg, a German biochemist, in the 1920s. He observed that cancer cells displayed an unusual preference for glycolysis over oxidative phosphorylation, even in the presence of sufficient oxygen, leading him to propose that mitochondrial dysfunction might be a characteristic of cancer metabolism. Since then, the Warburg effect has been extensively studied and is considered a hallmark of cancer metabolism.
While the exact reasons behind the Warburg effect in cancer cells are not fully understood, it is believed to confer several advantages to cancer cells, including providing rapid ATP production to support their increased growth and proliferation, supplying biosynthetic intermediates for cell division, and enhancing resistance to oxidative stress. Moreover, the increased lactate production by cancer cells can create an acidic microenvironment that promotes tumor invasiveness and metastasis.
The Warburg effect has significant implications for cancer research and therapy. Targeting the altered metabolism of cancer cells is an area of active investigation in developing potential treatments, including drugs that selectively inhibit glycolysis or enhance the reliance on oxidative phosphorylation, aiming to exploit the metabolic vulnerabilities of cancer cells for therapeutic benefit.
Conclusions
Cellular respiration stands as a fundamental process that sustains life by efficiently converting nutrients into usable energy in the form of ATP. This intricate pathway plays a pivotal role in the energy metabolism of all living organisms, from simple prokaryotes to complex multicellular beings. The stages of aerobic respiration, starting from glycolysis and culminating in oxidative phosphorylation, work in harmony to extract the maximum energy from glucose, providing the vital fuel for cellular activities. However, in the context of cancer biology, the Warburg effect highlights the significant impact of mitochondrial dysfunction on cellular metabolism, leading cancer cells to preferentially rely on glycolysis, even in the presence of oxygen. Such adaptations have profound implications for tumorigenesis, metastasis, and therapeutic interventions. Understanding the interplay between cellular respiration, mitochondrial function, and cancer provides valuable insights into potential targets for future therapies and opens new avenues for research in this complex and dynamic field.
Written by Rithika Suresh
Rithika Suresh completed her undergraduate degree in Biotechnology in Anna University before completing her masters in Biotechnology at University College Dublin.
Recent Posts
-
Growth Factors Can Cooperate to Promote Tumorigenesis
Introduction Tumorigenesis, the process by which normal cells transform into cancer cells …3rd May 2024 -
Inflammasome Activation Pathways: A Comprehensive Overview
Inflammasomes are complex intracellular structures that play a pivotal role in the immune respon …29th Apr 2024 -
Illuminating the Multifaceted Role of Acetylation: Bridging Chemistry and Biology Introduction:
Acetylation, a chemical process characterized by the addition of an acetyl functional group t …16th Apr 2024