Natural Killer Cells: A Guide
What are Natural Killer Cells?
Natural Killer (NK) cells are a vital subset of lymphocytes, belonging to the same family as B cells and T cells, collectively known as lymphocytes. They play a crucial role in the innate immune system, providing a rapid response against infected or cancerous cells without prior exposure or activation. NK cells are named "natural" because they can directly recognize and kill target cells without the need for prior sensitization or antigen presentation.
Natural Killer Cell
Function of Natural Killer Cells
NK cells exert their cytotoxic function through a multifaceted mechanism. Upon encountering a target cell, NK cells assess the balance of signals received through their activating and inhibitory receptors. If the activating signals dominate or the inhibitory signals are diminished, NK cells become activated.
Activated NK cells release the contents of their cytotoxic granules, which contain perforin and granzymes, in close proximity to the target cell. Perforin creates pores in the target cell's membrane, allowing granzymes to enter and initiate apoptosis. This process leads to the controlled and targeted elimination of the infected or cancerous cell.
In addition to their cytotoxic activity, activated NK cells can secrete various cytokines, including interferon-gamma (IFN-γ). IFN-γ plays a critical role in modulating the adaptive immune response by promoting the activation of other immune cells, such as macrophages and dendritic cells, further enhancing the immune response against infections or tumors.
NK cells also play a pivotal role in immunoregulation. Through interactions with various immune cells, such as dendritic cells and macrophages, they influence their activities and help maintain immune balance. Furthermore, NK cells can eliminate autoreactive T cells, promoting self-tolerance and preventing autoimmune responses. NK cells also exhibit antibody-dependent cellular cytotoxicity (ADCC), recognizing and destroying target cells coated with antibodies through Fc receptors.
Certain NK cells show memory-like properties called adaptive NK cells, responding more effectively upon re-encountering specific antigens. During pregnancy, NK cells support maternal-fetal tolerance, contributing to vascular remodeling and tissue development at the maternal-fetal interface.
NK Cells vs B Cells and T Cells
NK cells, T cells and B cells are types of white blood cells that play a role in the immune response. The main difference between NK cells and T cells is their function. NK cells are cytotoxic, meaning they can directly kill infected or damaged cells, while T cells help regulate the immune response and produce antibodies. Meanwhile, NK cells are part of the innate immune system, rapidly targeting infected or damaged cells, and B cells are part of the adaptive immune system, producing antibodies in response to specific antigens.
NK cells are essential for defending the body against viruses and cancer, whereas T cells play a crucial role in protecting against bacterial and other pathogenic infections. B cells contribute by producing antibodies that specifically target and neutralize pathogens during an immune response.
NK Cells | B Cells | T Cells |
Innate immune system |
Adaptive immune system |
Adaptive immune system |
Rapid, non-specific defense |
Antibody production |
Antigen recognition |
No immune memory |
Memory B cells |
Memory T cells |
Origins and Development of NK Cells
Natural Killer cells arise from a common lymphoid progenitor cell within the bone marrow. Hematopoietic stem cells, in the presence of specific cytokines, give rise to the lymphoid progenitor cells that eventually differentiate into mature NK cells. The development of NK cells can be broadly divided into several stages: commitment to the NK cell lineage, terminal maturation, and functional education.
During the commitment stage, key transcription factors such as Id2, E4BP4, and T-bet determine the lineage fate of lymphoid progenitor cells towards becoming NK cells. Following the commitment stage, the progenitor cells undergo terminal maturation to acquire the characteristic features of mature NK cells. This process involves the expression of various surface receptors, including CD56 and CD16 (FcγRIIIa), while losing expression of CD3, the marker for T cells. The maturation of NK cells is a tightly regulated process that ensures the acquisition of cytotoxic capabilities while maintaining self-tolerance.
The development of NK cells can be further detailed into distinct stages, based on cell surface expression. Stage 1 starts with pre-NK cell precursors (NKPs) arising from hematopoietic stem cells in the bone marrow. Unique to NKPs is their ability to differentiate exclusively into mature NK cells. Stage 2 further divides into two subsets, Stage 2a and Stage 2b, based on the expression levels of CD117 (c-Kit) and interleukin 1 receptor 1 (IL-1R1). As development progresses, Stage 3 marks the immature NK cells (iNKs), expressing higher levels of IL-1R1 and key activating receptors like NKG2D, CD335 (NKp46), CD337 (NKp30), and CD161 (NK1.1).
The subsequent stages, Stage 4a and Stage 4b, involve the transition from iNKs to mature NK cells. During these stages, the expression of various markers, including NKp80, NKG2D, CD335, CD337, and CD161, reaches peak levels. Stage 5 represents the full maturation of NK cells, characterized by the predominant expression of CD56dim and the initiation of CD16 (FcγRIIIA) and killer immunoglobulin-like receptor (KIR) (CD158) in subsets of NK cells. The final stage, Stage 6, is defined by the generation of 'adaptive' or 'memory-like' NK cells expressing high levels of NKG2C.
NK Cell Markers Expressed During Development
NK Cell Structure and Receptors
Natural Killer cells possess distinct structural features that facilitate their effector functions. Morphologically, NK cells are large granular lymphocytes characterized by abundant cytoplasm and a large nucleus. They contain cytotoxic granules in the cytoplasm that store perforin and granzymes, which are crucial effectors of NK cell-mediated cytotoxicity.
NK cells express a diverse array of cell surface receptors that play a pivotal role in target cell recognition and NK cell activation. Two major types of receptors are found on NK cells: activating receptors and inhibitory receptors. The activating receptors, such as NKG2D, NKp46, NKp30, and NKp44, engage stress-induced ligands on target cells or viral ligands and trigger NK cell activation. In contrast, inhibitory receptors, like killer cell immunoglobulin-like receptors (KIRs) in humans and Ly49 receptors in mice, recognize MHC class I molecules on healthy cells. The balance between activating and inhibitory receptor signaling determines the outcome of NK cell responses against target cells.
NK Cell Activation and Regulation
NK cell activation is a tightly regulated process governed by the integration of signals from activating and inhibitory receptors. This intricate balance ensures that NK cells can identify and eliminate infected or cancerous cells while avoiding unwarranted attacks on healthy cells. Various factors influence NK cell activation:
-
Loss of MHC Class I Molecules: Healthy cells express MHC class I molecules on their surface, which interact with inhibitory receptors on NK cells, providing a signal for self-recognition and preventing NK cell activation. However, virus-infected or cancerous cells may downregulate MHC class I expression as an immune evasion strategy. This loss of MHC class I molecules diminishes the inhibitory signal and tips the balance toward NK cell activation.
-
Activation Receptor Engagement: Activation receptors on NK cells recognize stress-induced ligands expressed on infected or transformed cells. These ligands can be induced during cellular stress, viral infection, or cellular transformation. When activation receptors engage their ligands, they provide positive signals that trigger NK cell activation and cytotoxicity.
-
Cytokine-Mediated Activation: In addition to receptor-mediated activation, NK cells can be stimulated by cytokines. Interleukin-2 (IL-2), interleukin-12 (IL-12), and interleukin-15 (IL-15) are essential cytokines that promote NK cell proliferation, survival, and activation. These cytokines are produced by other immune cells in response to infections and play a crucial role in enhancing NK cell activity.
Interactions with Other Immune Cells
NK cells engage in dynamic interactions with various immune cell populations, influencing the overall immune response and contributing to immune regulation. Some key interactions include:
-
Dendritic Cells (DCs): NK cells can interact with DCs, which are crucial antigen-presenting cells. Reciprocal interactions between NK cells and DCs can influence DC maturation and antigen presentation, shaping the adaptive immune response. By influencing DC function, NK cells help to orchestrate the adaptive immune response against specific pathogens.
-
Macrophages: NK cells play a critical role in the first line of defense by modulating macrophage activity through the secretion of IFN-γ. Upon encountering infected or abnormal cells, NK cells release IFN-γ, which activates macrophages, enhancing their phagocytic and microbicidal activities. This coordinated response between NK cells and macrophages helps to quickly eliminate potential threats and prevent the spread of infections.
-
T Cells: NK cells and T cells share some common features, such as the expression of activating receptors and the ability to secrete cytokines. Cross-talk between NK cells and T cells can occur during infections or inflammatory responses, reinforcing immune activation and promoting a coordinated response against foreign invaders.
- Tumor-Infiltrating Lymphocytes (TILs): In the context of cancer, NK cells are part of the TIL population that infiltrates the tumor microenvironment. NK cells can directly recognize and eliminate cancer cells, contributing to the immune surveillance against tumors.
NK Cells in Health and Disease
Natural Killer cells play a critical role in both maintaining health and combating various diseases. Their innate ability to recognize and eliminate infected or cancerous cells makes them essential components of the immune system.
- Defense Against Viral Infections:
- Cancer Surveillance and Immune Surveillance:
-
- NK cells play a critical role in immune surveillance against cancer. They can recognize and eliminate tumor cells that downregulate MHC class I molecules or express stress-induced ligands.
- Cancer cells may evade NK cell attack through various mechanisms, such as reducing ligand expression or inducing immunosuppressive factors.
-
- Autoimmune Diseases:
-
- NK cells are involved in regulating autoimmune responses. An imbalance between activating and inhibitory signals may lead to NK cell-mediated tissue damage in autoimmune diseases.
- In certain autoimmune conditions, such as rheumatoid arthritis and multiple sclerosis, NK cells' role in the pathogenesis is an active area of research.
-
- Immunodeficiency and NK Cell Dysfunction:
-
- Deficiencies in NK cell function or numbers can lead to increased susceptibility to viral infections and certain cancers.
- Primary immunodeficiency disorders affecting NK cells, such as familial hemophagocytic lymphohistiocytosis (HLH), are associated with severe immune dysregulation.
-
- Transplantation and Graft-versus-Host Disease (GVHD):
-
- NK cells are crucial in controlling graft-versus-host disease in allogeneic hematopoietic stem cell transplantation.
- The "KIR-ligand mismatch" between donor and recipient can influence the risk of GVHD and transplantation outcomes.
-
- NK Cell-Based Immunotherapy:
-
- Recent advances in immunotherapy have led to the development of NK cell-based therapies for cancer treatment.
- Approaches include adoptive transfer of ex vivo expanded autologous or allogeneic NK cells and the use of chimeric antigen receptor (CAR) NK cells.
-
NK Cells Related Kits
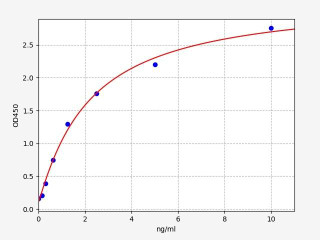
Human CD244 (Natural killer cell receptor 2B4) ELISA Kit (HUFI04564) | |
---|---|
ELISA Type | Sandwich |
Sensitivity | 0.094ng/ml |
Range | 0.156-10ng/ml |
Written by Sean Mac Fhearraigh
Seán Mac Fhearraigh PhD is a co-founder of Assay Genie. Seán carried out his undergraduate degree in Genetics at Trinity College Dublin, followed by a PhD at University College Dublin. He carried out a post-doc at the Department of Genetics, University of Cambridge. Seán is now Chief Technical Officer at Assay Genie.
Recent Posts
-
Inflammasome Activation Pathways: A Comprehensive Overview
Inflammasomes are complex intracellular structures that play a pivotal role in the immune respon …25th Apr 2024 -
Illuminating the Multifaceted Role of Acetylation: Bridging Chemistry and Biology Introduction:
Acetylation, a chemical process characterized by the addition of an acetyl functional group t …16th Apr 2024 -
Understanding IgA Test: Importance, Procedure, and Interpretation
The IgA test, also known as immunoglobulin A test, is a diagnostic tool used to measure the l …15th Apr 2024